
Development and applications of neuronal tracers
Dr. Elena Giusto
Our understanding of neuroanatomy has come a long way since Golgi and Cajal identified the morphology of isolated neurons for the very first time. Ever since, advances made in neighbouring disciplines have provided scientists with tools and techniques of unprecedented potential. In particular, the introduction of novel tracing reagents as well as developments of microscopes and image analysis software, have enormously contributed to the progressive unwiring of the central nervous system connections. In this article we will briefly outline some of the most popular tracers used in neuroanatomy, spanning from the classical horseradish peroxidase (HRP) to the newest viral-based transneuronal techniques.
Back and forth
The era of neuronal tracing began with two main findings: the discovery that damaged terminals undergo Wallerian degeneration (a progressive deterioration of the fibers from the periphery to the soma) and the observation that lesioned neurons are more susceptible to silver impregnation compared to healthy ones [1]. This not only allowed the identification of new anatomical details (e.g. terminal boutons) but also increased the awareness that neurons were endowed with an intrinsic ability to transport molecules to the periphery, essential for the survival of neuronal terminals. Later on, this intracellular traffic was shown to be bidirectional: molecules could be transported from the periphery to the soma (retrograde transport) and from the soma to the periphery (anterograde transport). This simple but crucial intuition set the basis on which a whole generation of “classic” and viral tracers has been developed in the past century (for extended reviews on the topic, please see [2-5]).
Old but gold: retrograde and anterograde tracers
One of the first approaches of neuronal tracing based on intracellular transport was introduced in the early 60s and relied on the delivery of radiolabelled amino acids [6]. Once incorporated by the endogenous ribosomal machinery, the distribution of neo-synthesized proteins - and so the morphology of the cell - would have been revealed by means of autoradiography. Although innovative and reliable, this protocol was soon replaced by more practical techniques.
Retrograde tracers
The introduction of HRP in 1971 marked a real milestone in the history of neuronal tracing [7]. HRP is a small enzyme that can be taken up via bulk endocytosis by peripheral terminals (e.g. following its injection on the gastrocnemius muscle) and retrogradely transported to the soma. Thanks to its enzymatic activity, HRP can react with hydrogen peroxidase and diaminobenzidine (DAB), resulting in a coloured product that can be easily detected with a light microscope. However, some peroxidase activity is naturally present within the cells; therefore, an initial quenching step is required. Despite still being widely used, HRP shows some technical limitations, such as the poor rate of peripheral uptake and the low degree of detail, with the visualization mainly restricted to the soma and the major dendritic arborizations.
Both uptake and transport were subsequently improved by conjugating HRP with either wheat germ agglutinin (WGA-HRP) or with the non-toxic B fragment of cholera toxin (CTB-HRP). Both WGA and CTB are internalized by cells via receptor-mediated endocytosis and have also been used as tracers on their own (either as plain tracers or conjugated with fluorophores), exhibiting both retrograde and anterograde properties, which are partially transferred to the conjugated counterparts as well. Importantly, this strategy allowed the replacement of colorimetric assays with more sensitive immunohistochemical protocols. One aspect that needs to be taken in consideration when choosing the optimal tracer(s) is the different distribution and/or affinity of receptors (e.g. higher for WGA in small sensory neurons) that may exist in different regions of the nervous system.
The introduction of inorganic fluorescent tracers represented another important novelty. Characterized by a prevalent retrograde transport, long-term stability, low toxicity and ease of detection, these tracers are often the first choice for multi-labelling protocols aimed at identifying the connectome of specific neuronal populations. Among the most used are Fluorogold, Fast Blue, Diamidino Yellow and the carbocyanines DiI and DiO. Likewise, fluorescent latex microspheres of different sizes and supplied with different coating materials, such as gold, have been widely employed. The latter not only can be morphologically distinguished in multiple labelling experiments, but are also suitable for fluorescent and electron microscopy applications.
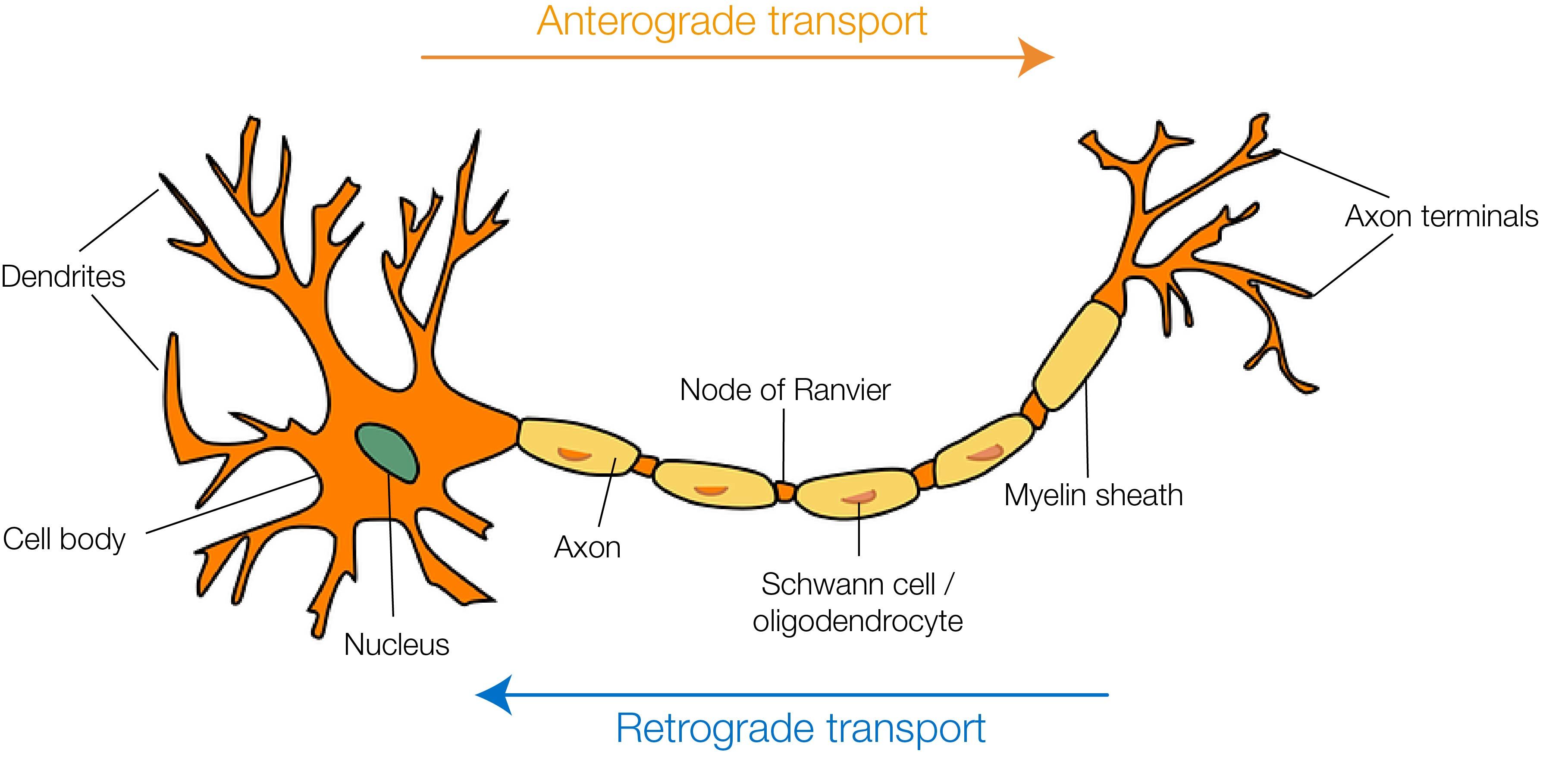
Anterograde tracers
Introduced in the mid 80s’, the Phaseolus vulgaris-leucoagglutinin (PHA-L) has been one of the first and still most widely used tracers to analyze efferent connections [5]. Similarly to WGA, PHA-L is internalized upon binding to a membrane receptor. PHA-L offers several advantages, among which the lack of background levels, since it is not naturally present in cells, the univocal transport direction and the ability to completely fill the cell, thus providing a very detailed, Golgi-like staining [8].
In the early 90s’, several dextran amines (DAs), among which biotinylated dextran amine (BDA), initially used as retrograde tracers, were discovered to follow an anterograde path as well. Now, DAs of different molecular weights and/or conjugated with different fluorophores are among the most popular tracers, thanks to their ability to reveal fine point details and their suitability to multi-labelling tracing protocols.
Finally, Biocytin (biotin conjugated to lysine) and Neurobiotin (N-(2-aminoethyl)biotinamide) have been used as valid anterograde tracers in protocols combining morphological and functional studies [9], such as electrophysiology. Both of these molecules are characterized by a short survival time, an aspect that limits their application to the study of short-range connectomes.
Considerations of classic tracers
Safe and effective, traditional tracers offer a good option to study interneuronal connections. However, some limitations must be considered before starting a new experiment. In particular, when combining multiple tracers, the experimenter needs to ensure that their fluorescent spectra do not overlap, and that the timing of injection(s) is skilfully calculated. For example, anterograde tracers are usually slower than retrograde ones. The amount of reagent inoculated also needs to be well-balanced; neither too low, which would lead to a weak signal, nor too high, which could result in cytotoxicity. Finally, some of the major flaws connected with the use of traditional tracers relate to the impossibility of marking specific neuronal populations, unless paired with suitable immunohistochemistry, and the lack of trans-synaptic tracing. Most of these issues have been overcome with the introduction of viral tracers, which have been popular in the past 20 years.
Viral tracers
By nature, neurotropic viruses are able to access the nervous system, replicate and spread to synaptically connected neurons. Compared to traditional tracers, viruses are not taken up by passage fibers, which does lead to some ambiguity in the final analysis, and require a smaller volume of injection. However, they are still considered a biological hazard and thus necessitate appropriate manipulation and facilities to guarantee the respect of the required biosafety level. Among the most popular viruses are the Herpes simplex virus type 1 (HSV-1), the human immunodeficiency virus (HIV), the adenovirus and the family of adeno-associated viruses (AAVs). These viruses have several differences in their characteristics, including their genome size and composition (DNA vs RNA), their ability to integrate in the host genome, their specific tropism, the titre needed for sufficient infection, their capsid composition, their toxicity and ability to replicate and spread trans-synaptically. The choice of virus therefore depends on the specific experimental design. As an example, lentivirus may be preferred when infecting neurons, thanks to its ability to integrate into post-mitotic neurons, gamma-retroviruses are optimal for lineage-tracing experiments, due to their ability to replicate in mitotic cells, AAVs are preferred for long-term experiments due to their low toxicity and long-term stability, while adenoviridae represent the best option when the experimenter needs a strong expression of their favourite transgene [2-3].
Thanks to major advances made in the field of molecular biology in recent years, we can now manipulate some of the viruses’ most harmful features and use them to our advantage. As an example, molecules of the capsid can be modified to restrict the entry of the virus to one or few specific subpopulations of neurons, or to univocally dictate its tracing direction (retrograde vs anterograde). We can modify the virus genome and replace some of its genes with sequences encoding for specific, promoter-driven reporters (e.g. GFP, eGFP) or functional molecules (e.g. channelrhodopsins) to modulate the activity of infected neurons. Also, viruses can be modified to study functional synaptic connections by using systems that express GFP only when the pre- and post-synaptic terminals come into contact [10]. We can also obtain viruses that can replicate their genome, and so amplify the signal to noise ratio, but cannot spread, thus avoiding the risk of uncontrolled proliferation. This has been possible by exploiting the lack of glycoprotein G expression in the rabies virus. Indeed, viruses depleted of this molecule cannot infect surrounding cells, allowing monosynaptic labelling, which is useful when the labelling of higher order neurons is not desired. At the same time, a promoter-specific expression of glycoprotein G can be achieved, either through co-infection or by using transgenic mice, thus limiting the viral spreading to a very restricted population of interest. These are just a few examples of the countless ways viruses have been applied so far.
Progression in the field of neuroanatomy has been fast and furious. Over the course of one century we have moved from the golden age of Golgi staining to the use of traditional and viral tracers. The efforts so far have been worth it: the advancement made in our understanding of the central nervous system connectome has been sensational and we can bet that, with all the tools available now, the best still has to come!
References
- Fink RP, Heimer L., 1967. Two methods for selective silver impregnation of degenerating axons and their synaptic endings in the central nervous system. Brain Res 4:369-374.
- Lanciego J.L, Wouterlood F.G, 2020. Neuroanatomical tract-tracing techniques that did go viral. Brain. Struct. Funct. 225(4):1193-1224.
- Nassi JJ, Cepko CL, Born RT, Beier KT. Neuroanatomy goes viral! Front Neuroanat. 2015; 9:80.
- Saleeba C, Dempsey B, Le S, Goodchild A and McMullan S, 2019. A Student’s Guide to Neural Circuit Tracing. Front. Neurosci. 13:897. doi: 10.3389/fnins.2019.00897.
- Lanciego J.L, Wouterlood F.G, 2011. A half century of experimental neuroanatomical tracing. J. Chem. Neuroanat. 42(3):157-83.
- Cowan et al,1972. The autoradiographic demonstration of axonal connections in the central nervous system. Brain Res. 37:21-51.
- Kristensson, K., and Olsson, Y.,1971. Retrograde axonal transport of protein. Brain Res. 29, 363–365.
- Gerfen CR, Sawchenko PE, 1984. An anterograde neuroanatomical tracing method that shows the detailed morphology of neurons, their axons and terminals: immunohistochemical localization of an axonally transported plant lectin, Phaseolus vulgaris leucoagglutinin (PHA-L). Brain Res 290:219–238.
- King MA, Louis PM, Hunter BE, Walker DW. 1989. Biocytin: a versatile anterograde neuroanatomical tract-tracing alternative. Brain Res 497:361-367.
- Feinberg, E. H., Vanhoven, M. K., Bendesky, A., Wang, G., Fetter, R. D., Shen, K., et al., 2008. GFP reconstitution across synaptic partners (GRASP) defines cell contacts and synapses in living nervous systems. Neuron 57, 353–363.
About the Author
Elena received her PhD in Experimental Neuroscience at the San Raffaele Institute, Italy. She then moved to Cambridge (UK) for her postdoc and finally to Brighton (UK) to work as a lab technician. Her main interest has been focused on translational neuroscience, and she has been working with stem cells and animal models of neurodegenerative diseases, including multiple sclerosis and spinal cord injury.