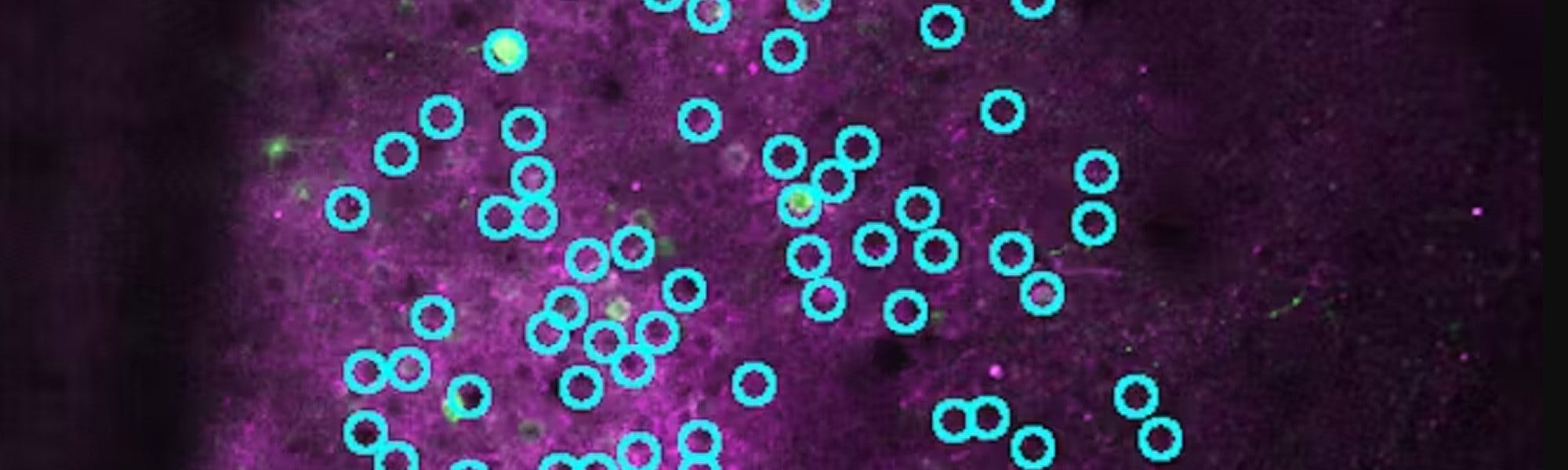
Precision Control: Advances in Holographic Stimulation for Two-Photon Optogenetics
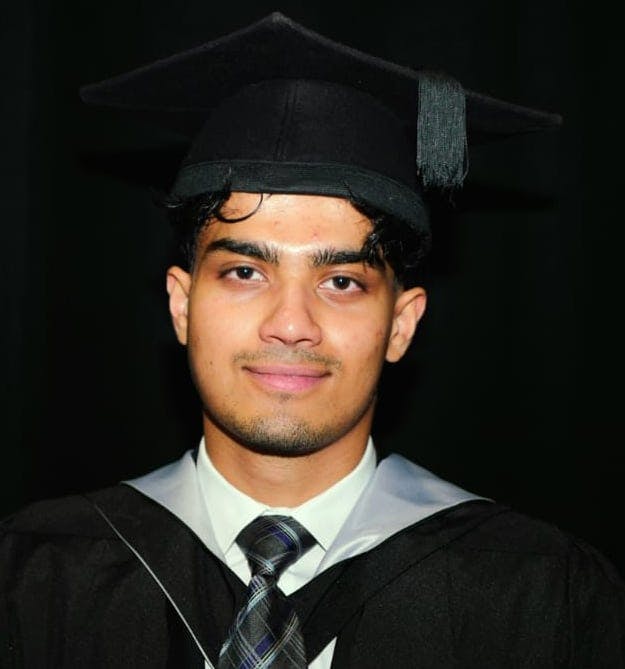
by Subham Ganguly, Institute of Psychiatry, Psychology & Neuroscience, King's College London
Introduction
Earlier this year, Filipe Machado Ferreira aptly likened the brain to "the harmonious coordination of an orchestra in full performance," a metaphor that vividly captures the intricate symphony of neural activity. Just as an orchestra employs polyrhythm—where multiple rhythms, each with distinct beats, weave together into a cohesive soundscape—the brain’s networks generate complex, overlapping spatiotemporal patterns. These patterns emerge from the unique interplay of neural circuit structures, shaped by intrinsic morphology, physiological properties, and the adaptive transformations occurring over a lifetime of experiences and stimuli. Each "note" of neural firing builds upon others, enabling the finely tuned coordination required for processing information seamlessly each day.
Understanding the interactions between neurons within a neural circuit is central to the appeal of optogenetics. In vivo calcium imaging provides an indirect yet insightful readout of neural activity1, while channel rhodopsin-based photoactivation enables precise manipulation of neuronal firing2,3 (Figure 1a). Through this approach, researchers can activate or inhibit specific neurons by exploiting cellular mechanisms and ion gradients, allowing them to observe the effects within the context of an interacting circuit. By combining in vivo calcium imaging with targeted photo stimulation, scientists can perturb individual neurons within a circuit under physiological conditions, uncovering the fundamental mechanisms and computational processes that support local circuit dynamics. This approach provides an unprecedented window into how neural networks coordinate the large-scale transfer of information across space and time, illuminating the sophisticated patterns that drive coherent brain activity.
Figure 1 . (a) Expression of a calcium indicator and a stimulatable opsin within a cranial window, demonstrating the ability to target a large number of cellular populations simultaneously. The co-expression highlights the potential for both activity monitoring and precise manipulation of neural circuits within the same field of view. (b) Schematic of the imaging and stimulation laser pathway in an SLM-integrated two-photon microscope setup. The diagram illustrates how the spatial light modulator (SLM) is used to phase-modulate the stimulation laser, enabling simultaneous multi-cell targeting, while the imaging laser captures calcium-related neural activity, allowing for real-time all-optical interrogation.
Balancing Spatial and Temporal Precision: Challenges and Advances in Two-Photon and One-Photon Approaches
Optogenetics offers visual readouts of neural activity, but these signals must still be imaged to enable spatially and temporally precise analysis at the cellular level. Two-photon imaging has advanced this precision (Figure 1b), allowing researchers to capture calcium-related activity with spatial accuracy down to individual spines. This enables detailed mapping of cellular activity at remarkable scales. However, optogenetics faces temporal limitations, primarily due to the dynamic response times of optogenetic indicators. While recent generations of calcium indicators have greatly improved in response speed, they still lag behind the temporal resolution of electrophysiological methods.
While two-photon perturbation of neural circuits has opened new possibilities for spatial precision in all-optical interrogation, its application remains limited by the small scale of perturbation achievable at any given moment. Although the spatial specificity of two-photon imaging is a significant advantage, the precision of two-photon beams restricts the area that can be simultaneously targeted and perturbed. This high spatial resolution confines influence to small, localized regions of the neural circuit, posing challenges for studies requiring broader or more complex activation patterns4.
Given a dwell time of approximately 2 milliseconds, which is standard in all-optical two-photon stimulation of single cells, even with near-instantaneous repositioning of the two-photon beam using galvanometer mirrors, targeting up to 100 different cells sequentially would take 100–200 milliseconds. This duration limits the temporal precision needed to capture rapid, large-scale circuit dynamics. Consequently, the field has increasingly adopted a hybrid approach, combining two-photon imaging with one-photon perturbation. This strategy enables the simultaneous stimulation of thousands of cellular targets within a single 2-millisecond time window, facilitating larger, circuit-level perturbations across the entire field of view and overcoming the temporal and spatial constraints of purely two-photon approaches.
The Role of Spatial Light Modulators in Precision Neural Circuit Perturbation
Returning to the concept of brain networks as complex, overlapping spatiotemporal patterns—each with its own rhythms and distinct beats—an unbiased perturbation of multiple circuits across a large area is unlikely to achieve the physiological specificity needed to distinguish intricate differences in circuit-specific neural activity. Although stimulation power and frequency can be carefully controlled, all neurons expressing channelrhodopsins are subject to the same perturbation dynamics, potentially overlooking the unique, rhythmically nuanced interactions within each circuit. This lack of specificity underscores the challenge of precisely tuning perturbations to match the natural complexity of individual networks.
Enter the application of spatial light modulators (SLMs) in microscopy—a ground-breaking system capable of phase-modulating a laser beam to generate any spatiotemporal light pattern, enabling simultaneous illumination of multiple selected regions of interest5,6. This technology allows a single 2-photon beam to be split into spatial patterns, targeting numerous channelrhodopsin-expressing cells within a field of view, with each coordinate receiving light with 2-photon specificity7.
An SLM, such as Meadowlark’s 1024x1024 model, operates through an array of rotatable liquid crystal beads that adjust their orientation and angle based on applied voltage, generating precise spatial light patterns (Figure 2). This device can simultaneously target up to 375 distinct locations within a ~200 µm column of tissue. When combined with galvanometer mirror movements, the effective field of view extends to ~600 µm through sequential stimulation. The system supports 2-photon calcium imaging alongside spatially patterned photostimulation, with an SLM refresh rate of 1.7 milliseconds and galvanometer repositioning within 3 milliseconds. Together, these capabilities allow the activation of thousands of neurons within a 10-millisecond window, delivering unparalleled precision and scalability in all-optical neural circuit interrogation.
Figure 2: Spatial Light Modulator (SLM) Functionality and Considerations - (a) Illustration of an SLM splitting a single laser beam into multiple, spatially precise targets. The figure demonstrates how phase modulation enables simultaneous targeting of specific cellular regions within the field of view. (b) Etchings on a microscope slide, showing the capability of SLM-integrated systems to create complex spatial patterns and structures. The integration with galvanometer mirror movements further highlights the flexibility to adjust targeting dynamically across a larger area. (c) Diagram of the diffraction limit and laser etchings across an SLM field, illustrating how power distribution varies spatially across the modulator. This highlights the necessity of power tuning to account for uneven illumination and to ensure optimal stimulation of cells in specific regions of the SLM’s field.
Designing Functionally Meaningful Experiments with Spatial Light Modulators
So, we’ve established that spatial light modulators are game-changing—they allow researchers to harness the spatial specificity of a two-photon beam while achieving the temporal precision needed to target thousands of cells in a remarkably short span of time. An engineer can be called, a spatial light modulator purchased, calibrated, and integrated into your two-photon microscopy setup. But what comes next?
How do we effectively target cells within our region of interest in the brain? What features should guide the grouping of cells we choose to stimulate? And, most importantly, how do we design experiments to intentionally probe neural networks and uncover the dynamics that are most crucial for the function of a given brain region? These questions lie at the heart of leveraging this technology for meaningful insights, bridging the gap between technological capability and neuroscientific discovery.
Targeting cells in a functionally meaningful way can vary significantly depending on the cell type, circuitry, and brain region of interest. However, I can provide a few starting points and considerations when it comes to perturbing cellular targets.
What role does your cell type play in your brain region of interest? By expressing calcium indicators and channelrhodopsins specifically in the neuronal subtype you’re studying, you can perturb and probe the activity profiles of these cells to understand how their function leads to downstream changes. These changes can cascade through the circuit and network, ultimately affecting biological processes or behavioral outcomes.
For example, chandelier cells in the hippocampus exert precise GABAergic inhibition on pyramidal neurons by targeting their axon initial segment (AIS)8. This specialized interaction enables chandelier cells to tightly regulate the firing threshold of pyramidal neurons, thereby controlling the timing and synchronization of their activity. This synchronization is thought to be critical for hippocampal functions such as memory encoding and retrieval. By perturbing chandelier cells, researchers can explore their role in modulating circuit dynamics and examine how their activity contributes to the temporal coordination necessary for memory processes.
Similarly, equivalent experiments could be conducted in the visual cortex to investigate neurons tuned to specific features of visual stimuli. By perturbing neurons known to activate in response to particular orientations, contrasts, or features during an active discrimination task, one could uncover their feedforward roles in relaying visual information to decision-making centers in the brain9. Such experiments would shed light on how these neurons contribute to feature extraction and how this information integrates into broader neural computations guiding perception and action.
Slightly biased by my own interest in the cerebellum and the role Purkinje cells play in shaping cerebellar output to distant cortical regions, another prime target for perturbation is the firing of Purkinje cells during prediction-error paradigms. These experiments could explore how the cerebellum adapts its activity in response to an ever-evolving understanding of the world and its rules. By perturbing Purkinje cell activity, researchers can investigate whether this adaptation influences far-reaching cortical regions, potentially driving behavioral adjustments (Figure 3). Such studies could provide valuable insights into how cerebellar computations contribute to the integration of predictive and error signals, ultimately shaping behavior in dynamic environments10.
Figure 3: Microzone-Specific Encoding of Forepaw Movement and Reward in the Cerebellum (a) Schematic representation of a behavioral paradigm from a study by Dimitar Kostadinov, where mice were trained to perform a wheel-turning task. The task design facilitated the investigation of cerebellar neural activity associated with forepaw movement and reward. (b) Neural activity recordings from the cerebellum, showing distinct encoding of forepaw movement and reward signals. These signals were mapped to neighboring microzones of Purkinje cells, highlighting the cerebellum's unique ability to structurally and functionally separate these representations. (c) Diagram illustrating the spatial organization of microzones within the cerebellum, emphasizing the localized yet distinct activity patterns associated with different behavioral components, underscoring the cerebellum’s role in integrating motor and reward-related computations.
Optimizing Stimulation Strategies for Functional and Physiological Relevance
Once a cell type of interest and an experimental paradigm have been established, it becomes crucial to understand the intrinsic functional properties of the cell type to design perturbation strategies that align with biologically relevant firing frequencies and dynamics. Two-photon stimulation beams are rarely exposed to tissue without some form of pulsing. For example, most SLM-enabled photostimulation setups inherently pulse at 266 femtoseconds, but further adjustments to parameters such as frequency of exposure, duration of exposure, and repetition count are often required to achieve a physiologically relevant stimulation profile.
These optimizations are critical to ensure that perturbations effectively mimic natural activity patterns and provide meaningful insights into the function and dynamics of the targeted neural circuits. For instance, chandelier cells, which are fast-spiking neurons, require trains of rapid action potentials at a rate of 40–200 Hz. In contrast, Purkinje cells fire at relatively slower rates of 20–100 Hz. These differences necessitate fundamentally distinct stimulation strategies, including variations in power output, duration of exposure, and repetition count of the beam activity. Tailoring these parameters to the specific firing dynamics of each cell type is essential for accurate and biologically relevant experimental outcomes.
Optimizations must extend beyond the firing properties of specific cell types to include their functional relevance. If your cell type exhibits temporally unique firing patterns—whether across a behavioral paradigm or during spontaneous activity—these patterns can be leveraged to create a functional mapping of cells.
In the cerebellum, for instance, Purkinje cells are organized into spatial structures known as microzones, which receive feedforward input from the same source neuron and relay coordinated output to the deep cerebellar nuclei. Functional metrics, such as calcium imaging, can be employed to identify these microzones. Research indicates that Purkinje cell dendrites within a single microzone exhibit synchronized activity within the same temporal window, a characteristic that differentiates them from adjacent microzones11.
This functional organization highlights the necessity of tailoring stimulation strategies not only to the intrinsic firing properties of individual cells but also to the broader spatial and temporal dynamics of the circuits they belong to. By understanding the temporally unique roles of individual microzones relative to their neighbors, experimenters can group Purkinje cells into functionally relevant stimulation targets. This approach helps avoid the critical mistake of stimulating two functionally distinct microzones with a single perturbation, thereby preserving the integrity of circuit-specific investigations (Figure 4).
Figure 4: Functionally Relevant Perturbation Targeting (a) Identification of functionally relevant neuron groups based on neural activity patterns. The figure demonstrates how calcium imaging or electrophysiological recordings can reveal spatially and temporally coherent groups of neurons with specific functional roles. (b) Schematic of tailored perturbation design, showing the creation of targeted stimulation groups that align with identified functional relevance, enabling precise and meaningful circuit manipulation. (c) Comparison of functionally guided perturbation approaches, illustrating how targeted stimulation could potentially produce more consistent and biologically significant outcomes.
Interpreting Data: Computational Modelling and Circuit Dynamics
Now that you’ve established an SLM-enabled photostimulation protocol, set up your rig for the experiments, selected your animal model of interest expressing the appropriate neural activity indicators and channelrhodopsins, and successfully run experiments probing circuits with specific functional profiles and firing properties, the next question arises: How will you interpret the data? How do you determine whether your stimulation paradigms have led to meaningful and significant outcomes?
One promising avenue for interpreting data, beyond the traditional approach of statistical comparison of perturbed versus non-perturbed firing properties and calcium-related neural activity measures, is through computational modeling. Leveraging the principles of dynamical systems theory, which posits that a function describes the time-dependent trajectory of a point in the ambient space of neural activity, allows for a deeper exploration of circuit dynamics12.
By incorporating the rich temporal and spatial patterns of neural activity—captured through calcium indicators or electrophysiological recordings—these models can trace the evolution of neural activity over time. This approach enables researchers to investigate how changes in activity propagate through circuits and ultimately lead to biologically or behaviorally relevant outcomes. For instance, one could model the neural trajectories underlying a task13, such as discriminating between two visual stimuli, and identify how specific perturbations influence the neural computations responsible for successful task performance.
Understanding how perturbations drive neural circuits toward specific outcomes offers profound insights into the biologically relevant effects of your perturbation methods. It also deepens your understanding of the mathematical encoding and information relay performed by your specific cell type within its overarching circuit. By probing these dynamics, you can uncover how individual cell types contribute to circuit-level computations and how these computations translate into functional or behavioral outcomes, shedding light on the fundamental principles governing neural information processing.
If this piques your interest, I highly recommend exploring topics such as linear and non-linear dynamical systems, recurrent neural networks (RNNs), and latent dynamics — and their applications in computational and systems neuroscience. These areas provide powerful frameworks for modeling and understanding the temporal evolution of neural activity, uncovering hidden patterns in complex datasets, and linking neural computations to behavior. They offer valuable tools to interpret how neural circuits process, encode, and relay information within biological systems.
Real-Time Control and Future Directions in Neural Circuit Interrogation
Once you have developed a strong understanding of the neural dynamics in your system and the role your cell type of interest plays within these spatiotemporal dynamics, an exciting opportunity arises: performing real-time closed-loop control of your circuit’s activity14. By understanding the temporal evolution of neural activity and its relationship to specific biological or behavioral outcomes, you can design precise perturbations to influence these outcomes.
What types of perturbations could be applied, and at what time intervals, to drive the organism to choose or act differently? How consistently can these manipulations produce the desired changes? These questions lie at the frontier of neuroscience, allowing researchers to probe causality and explore the functional flexibility of neural circuits in real time.
The outcomes that feel most significant and relevant to me in this research lie in its potential applications, such as neuroprosthetics, brain-computer interfaces, and alleviating the effects of epileptic seizures through real-time prediction and closed-loop intervention. While these align closely with my personal interests, I am confident that there are countless other applications yet to be explored, waiting to be unlocked by the discovery of information from this technology.
I hope that through reading this, you have gained a clear starting point for conducting all-optical interrogations of neural circuits, including the application of SLM-enabled systems for single-cell-specific probing within larger, more complex neural networks. Moreover, I hope this has provided insight into the fundamental biological, behavioral, and computationally relevant outcomes you can explore using this powerful experimental protocol. With this approach at your disposal, the potential to unravel the intricate dynamics of neural circuits and their contributions to system-level functions is within reach.
About Subham Ganguly
Subham holds a bachelor's degree in Neuroscience from King's College London and a master's degree in Brain Sciences from University College London (UCL). Currently, he is in the first year of his PhD, where his research focuses on exploring the contributions of the cerebellum to far-range cortical brain regions and dynamics in the lab of Dr Dimitar Kostadinov.
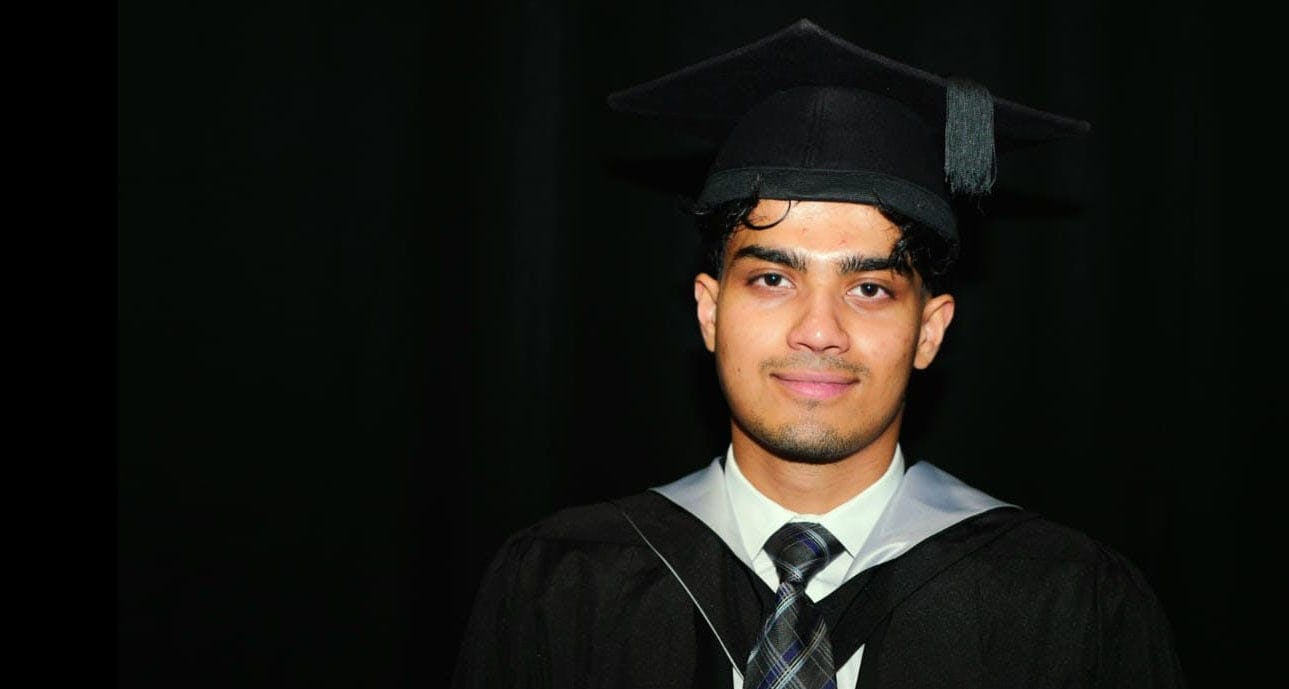
References
1. Zhang, Y., Rózsa, M., Liang, Y., Bushey, D., Wei, Z., Zheng, J., Reep, D., Broussard, G.J., Tsang, A., Tsegaye, G., et al. (2023). Fast and sensitive GCaMP calcium indicators for imaging neural populations. Nature 615, 884–891. 10.1038/s41586-023-05828-9.
2. Prakash, R., Yizhar, O., Grewe, B., Ramakrishnan, C., Wang, N., Goshen, I., Packer, A.M., Peterka, D.S., Yuste, R., Schnitzer, M.J., et al. (2012). Two-photon optogenetic toolbox for fast inhibition, excitation and bistable modulation. Nat. Methods 9, 1171–1179. 10.1038/nmeth.2215.
3. Kishi, K.E., Kim, Y.S., Fukuda, M., Inoue, M., Kusakizako, T., Wang, P.Y., Ramakrishnan, C., Byrne, E.F.X., Thadhani, E., Paggi, J.M., et al. (2022). Structural basis for channel conduction in the pump-like channelrhodopsin ChRmine. Cell 185, 672-689.e23. 10.1016/j.cell.2022.01.007.
4. Oron, D., Papagiakoumou, E., Anselmi, F., and Emiliani, V. (2012). Two-photon optogenetics. Prog. Brain Res. 196, 119–143. 10.1016/B978-0-444-59426-6.00007-0.
5. Packer, A.M., Russell, L.E., Dalgleish, H.W.P., and Häusser, M. (2015). Simultaneous all-optical manipulation and recording of neural circuit activity with cellular resolution in vivo. Nat. Methods 12, 140–146. 10.1038/nmeth.3217.
6. Russell, L.E., Dalgleish, H.W.P., Nutbrown, R., Gauld, O.M., Herrmann, D., Fişek, M., Packer, A.M., and Häusser, M. (2022). All-optical interrogation of neural circuits in behaving mice. Nat. Protoc. 17, 1579–1620. 10.1038/s41596-022-00691-w.
7. Linnenberger, A.M. (2018). Advanced SLMs for microscopy. In Adaptive Optics and Wavefront Control for Biological Systems IV, T. G. Bifano, S. Gigan, and J. Kubby, eds. (SPIE), p. 3. 10.1117/12.2290455.
8. Woodruff, A.R., Anderson, S.A., and Yuste, R. (2010). The enigmatic function of chandelier cells. Front. Neurosci. 4, 201. 10.3389/fnins.2010.00201.
9. Lee, S.-H., Kwan, A.C., Zhang, S., Phoumthipphavong, V., Flannery, J.G., Masmanidis, S.C., Taniguchi, H., Huang, Z.J., Zhang, F., Boyden, E.S., et al. (2012). Activation of specific interneurons improves V1 feature selectivity and visual perception. Nature 488, 379–383. 10.1038/nature11312.
10. Kostadinov, D., and Häusser, M. (2022). Reward signals in the cerebellum: Origins, targets, and functional implications. Neuron 110, 1290–1303. 10.1016/j.neuron.2022.02.015.
11. Kostadinov, D., Beau, M., Blanco-Pozo, M., and Häusser, M. (2019). Predictive and reactive reward signals conveyed by climbing fiber inputs to cerebellar Purkinje cells. Nat. Neurosci. 22, 950–962. 10.1038/s41593-019-0381-8.
12. Izhikevich, E.M. (2006). Dynamical systems in neuroscience: the geometry of excitability and bursting (The MIT Press) 10.7551/mitpress/2526.001.0001.
13. Russo, A.A., Khajeh, R., Bittner, S.R., Perkins, S.M., Cunningham, J.P., Abbott, L.F., and Churchland, M.M. (2020). Neural Trajectories in the Supplementary Motor Area and Motor Cortex Exhibit Distinct Geometries, Compatible with Different Classes of Computation. Neuron 107, 745-758.e6. 10.1016/j.neuron.2020.05.020.
14. Zhang, Z., Russell, L.E., Packer, A.M., Gauld, O.M., and Häusser, M. (2018). Closed-loop all-optical interrogation of neural circuits in vivo. Nat. Methods 15, 1037–1040. 10.1038/s41592-018-0183-z.